Gene Therapy Finds Its Groove
Successes in treating deafness, sickle cell disease, and cardiac amyloidosis show potential of recent advances
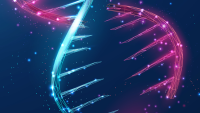
By Alan Dove, Portraits by Jorg Meyer
Since the dawn of molecular biology in the mid-20th century, scientists and physicians have dreamed of modifying human DNA to cure diseases. The field finally started to take off in the 1990s, when the first gene therapy clinical trials began, but early optimism by researchers and pharmaceutical companies soon collapsed in the face of a series of failures. Those setbacks culminated with the 1999 death of a subject in a gene therapy trial.
Although corporate interest in gene therapy plummeted, a handful of investigators, including several at Columbia, continued to work on it. In the past 20 years, researchers have slowly been figuring out why the earlier trials failed and developing new tools to overcome those problems. The serendipitous discovery of the CRISPR-Cas system in bacteria, which has since been modified into a molecular toolbox for high-precision gene editing, has invigorated the field even further.
A growing number of Columbia faculty are pushing gene therapy into its next phase. Some are running clinical trials that can cure or mitigate rare genetic diseases, while others are pursuing basic research to improve the safety of gene therapies and make them useful for a broader range of conditions.
The Sound of Success
Lawrence Lustig, MD, the Howard W. Smith Professor and Chair of Otolaryngology/Head & Neck Surgery, has been working on gene therapy long enough to have seen both its early lows and recent highs. As an otolaryngologist, his initial gene therapy project was an attempt to regenerate hair cells in human ears.
“Sound vibrations are processed by the hair cells into an electric impulse, which is then transmitted to the brain, and most cases of hearing loss come from the loss of those hair cells,” says Dr. Lustig. After scientists discovered the molecular signals that prompt hair cell growth during development, drug companies’ ears perked up.
In the early 2010s, Novartis sponsored a clinical trial that attempted to apply those findings in adults with hearing loss. “We were one of three sites to try to use gene therapy in humans to regrow hair cells in the ear; unfortunately, it was a spectacular failure,” says Dr. Lustig. Subsequent analysis suggested that adding a gene was necessary for hair cell growth, but adding a gene was not sufficient in the absence of other signals from adjacent supporting cells.
After that experience, otolaryngologists regrouped around the idea of treating rarer genetic forms of deafness, especially in children. They hoped that intervening earlier in life and targeting specific known molecular defects would bring more success. “There are over a hundred different individual genetic mutations that can lead to deafness that we’ve identified,” says Dr. Lustig. A few years ago, his laboratory found that a protein called otoferlin is necessary for carrying auditory signals from the ear to the brain. In patients with mutations in their otoferlin genes, that leads to profound deafness even though their ear structures are intact. That raised hope that repairing the genetic mutation would restore the missing piece for the children’s hearing. “It gives us a long window during childhood in which we can potentially intervene and restore normal otoferlin function—and get those kids to hear.”
Several companies have now jumped into gene therapy efforts targeting otoferlin, and Dr. Lustig is running one of the clinical trials at Columbia. The trial uses a genetically modified adeno-associated virus, a popular gene therapy vector that has the advantage of reliably integrating its genome—or an engineered target gene—into human DNA. Because the otoferlin gene is too big to fit inside the virus, though, the therapy’s developers have split it into two separate viral vectors that are delivered simultaneously.
Early results from the first patients in the trial, at a site in the UK, just came out this year. “Now we have data out to 24 weeks, and one previously deaf child has auditory thresholds in the normal range. It’s really remarkable,” says Dr. Lustig. Besides changing individual patients’ lives, the results are also revitalizing the entire field. “Now that we’ve got a big win, it’s going to drive a lot of funding into this space to help, and we hope to address more common forms of deafness.”
The Blood Line
While gene therapy often focuses on rare diseases because of their genetic tractability, at least one highly prevalent condition is also a promising target: sickle cell disease. Affecting an estimated 100,000 people in the United States alone, and many more worldwide, sickle cell disease stems from hemoglobin gene mutations that are especially common in people of West African descent. In individuals with two mutated copies of the gene, the condition can be debilitating and life-shortening.
Until recently, the only long-term cure for sickle cell was to eliminate the patient’s own blood-regenerating stem cells with powerful chemotherapy, then transplant new blood or bone marrow-derived stem cells of a closely matched family or unrelated donor. “That definitely has the potential of curing the patient, but at the end of the day it may have also given them graft versus host disease, which can also be a chronic condition,” says Markus Mapara, MD, professor of medicine and director of the bone marrow transplantation and cell therapy program at Columbia.
That problem drove Dr. Mapara to work on gene therapy for the condition, starting about 10 years ago. Dr. Mapara and his colleagues participated in clinical trials that took patients’ own blood stem cells and infected them with a genetically engineered lentivirus, which delivered a gene to express high levels of an engineered fetal hemoglobin-like b-globin. They eliminated the rest of the patients’ stem cells with chemotherapy before reintroducing the modified stem cells. Fetal hemoglobin interferes with the protein polymerization that leads to sickle cell crises, in which the hemoglobin polymers deform red blood cells into sickle shapes, causing them to clog in the circulatory system with painful, life-threatening consequences.
“Those trials have been enormously successful, and eventually they led to the approval of the treatment by the FDA in December of last year,” says Dr. Mapara. A competing therapy, which aims at re-expressing actual fetal hemoglobin but uses a CRISPR-based gene editing system instead of a lentiviral vector, won approval at the same time. Columbia also participated in that trial, and Dr. Mapara says he considers the two treatments equivalent. “It’s very difficult at this point to make a suggestion to patients; you cannot really recommend one treatment over the other.”
In both cases, the biggest drawback is the chemotherapy needed to ablate the patient’s resident stem cells before transplanting the modified cells back in. That procedure carries substantial risks, ranging from infertility to possible development of cancer. Dr. Mapara and his colleagues hope to address that. “Is it possible to get those genetically engineered cells in without the need for this high-dose chemotherapy? That is something which is actively being looked at by a number of groups,” he says.
Meanwhile, he is encouraged not only by the success and approval of the two new treatments, but also the long-term prospects for patients. “When you include the phase one and two trials, we have eight or nine years of follow-up for a lot of the patients, and it seems to be fairly safe and stable,” says Dr. Mapara.
Close to the Heart
Mathew Maurer, the Arnold and Arlene Goldstein Professor of Cardiology (in Medicine), also uses gene editing to address a problem that disproportionately affects certain racial and ethnic groups: cardiac amyloidosis. The condition occurs when defective molecules of transthyretin, a protein produced in the liver that carries vitamin A and a thyroid hormone to different parts of the body, clump together in cardiac tissue and damage the heart.
Dr. Maurer cites a long institutional tradition in studying transthyretin. “The protein was discovered at Columbia, the genetic sequence was determined at Columbia, and the first pathogenic variant was described here,” he says.
Over a hundred other genetic variants have since been described, in ethnic groups that hail from Portugal, West Africa, Ireland, and several other regions. Those variants predispose the protein to breaking apart and forming amyloid fibrils, but the disease also can arise from transthyretin with a normal genetic sequence, usually late in life.
Some drugs can stabilize transthyretin to prevent it from falling apart and aggregating, while others can inhibit its production entirely. Dr. Maurer and his colleagues have been testing a more durable approach, using a CRISPR-based gene editing therapy developed by Intellia. The treatment delivers the CRISPR-Cas editing system with a guide RNA that targets the transthyretin gene to disable its expression in the patient’s liver cells. “The advantage of gene editing is that it’s a single infusion, and it’ll knock down and permanently stop your liver’s ability to make this protein,” says Dr. Maurer, international co-PI on the CRISPR trial and co-chair of the trial’s steering committee. The trial is the first to reach phase 3 with an approach that fixes a patient’s genes in situ.
Stopping expression of an important protein sounds risky, but it turns out that transthyretin isn’t essential. “When you shut off the protein’s production, you have many other proteins that can carry your thyroid hormone, and those can seemingly make up for the loss. However, patients must take a daily vitamin A supplement to make up for the loss of the protein’s ability to transport vitamin A,” says Dr. Maurer. Patients on an earlier transthyretin-inhibiting drug have been doing well for several years. The phase 3 clinical trial of the new gene editing therapy is ongoing at many sites throughout the world, with Dr. Maurer leading the trial at Columbia.
Gene therapy could make cardiac amyloidosis treatment much more accessible. “Right now the only drug that’s approved specifically for cardiac amyloidosis is Tafamidis,” says Dr. Maurer. Costing nearly a quarter million dollars a year per patient, Tafamidis is the most expensive cardiovascular drug ever launched in the United States. “If you can give CRISPR gene therapy and then stop other very expensive therapies, it could turn out to be very cost-effective.”
Other cardiac gene therapies will likely be harder to develop, though. “The liver’s an easy target for current gene modifying and silencing-based therapies; the heart is much more difficult,” says Dr. Maurer. Genetically modified viruses are still the most promising way to target gene therapies to cardiac muscle, but pre-existing immune responses to those viruses often eliminate them before they have a chance to deliver their payloads.
System Update in Progress
The drawbacks of viral vectors have driven researchers to focus on newer gene editing strategies, which continue to improve. “Now that tools exist to specify with very high precision the site of a genetic modification, the early gene therapy methods—version 1.0, if you will—may become obsolete over time,” says Sam Sternberg, PhD, associate professor of biochemistry & molecular biophysics.
Indeed, the gene editing approach based on the bacterially derived CRISPR-Cas9 system has already undergone several rapid cycles of bug fixes and performance enhancements to make it even more powerful. Researchers have focused on reducing the system’s off-target effects, which can cause unwanted mutations in regions far from the targeted gene, and finding ways to deliver the gene editing machinery to specific tissues.
Meanwhile, Dr. Sternberg and other scientists in the field have been developing entirely new techniques that overcome some of the major limitations of CRISPR-Cas9 technology. “The early phase of results has now raised the bar, to where newer technologies should be able to perform modifications with better control and accuracy, with off-target effects being profiled from the beginning,” says Dr. Sternberg.
In one project, for example, Dr. Sternberg’s lab uses a different bacterially derived system, which the team calls CAST (for CRISPR-associated transposase), to insert large pieces of DNA into the genomes of cultured human cells. Unlike CRISPR-Cas9, which can make only small edits to a gene, CAST can be used to add entire genes. “We colloquially refer to this approach as gene therapy 2.0 because it still delivers healthy genes into the genome, but it’s now controlled by a guide RNA, which means we can specify where the genes end up,” he explains.
That approach could make gene therapy much more widely useful; instead of creating a different treatment for each mutation that causes a disease, therapy developers could devise a single treatment that replaces all defective versions of a mutated gene. “If there are only a couple hundred patients in the world with a given mutation, it may not be feasible to initiate bespoke clinical trials for such a small patient cohort. But if we could land the healthy gene in a specific region of the genome that would be therapeutically beneficial for all patients with the disease, regardless of their specific mutation, it would represent a much more potent and scalable strategy,” says Dr. Sternberg. He and his colleagues are currently working to do just that with the CFTR gene, which is mutated in patients with cystic fibrosis.
Other researchers around the world also have developed next-generation gene editing and guided gene insertion techniques in recent years, and Dr. Sternberg has been tracking the field’s progress closely. “In the end,” says Dr. Sternberg, “we care less about which particular technology is being used and more about what gets us to the finish line, which is making a therapeutic edit in an accessible and scalable way that is safe and effective for patients.”